A handful of amino acid mutations in an enzyme from an Antarctic octopus equips it with remarkable cold tolerance. The discovery came from comparing enzymes from a cold-water and a temperate-water species. Insight into the cold-adapted enzyme might have relevance for cryogenic preservation or organ transplantation.
Researchers in the US began by investigating the workings of a crucial Na+/K+ ion pump from an octopus that had been collected below the ice in McMurdo Station, Antarctica. This Na+/K+ ion pump, powered by ATP, pushes three sodium ions out for every two potassium ions in, generating the electrochemical gradient that is critical to allow neurons to fire. In neurons, the activity of this protein uses around three-quarters of a cell’s total energy output, notes lIya Levental, a molecular physiologist at the University of Virginia.
‘As we lowered the temperature, the ATPase from the Antarctica octopus kept working way faster than the temperate octopus [one], which pretty much stopped once we reached 8°C,’ recalls Miguel Holmgren at the National Institute of Neurological Disorders and Stroke in Bethesda, Maryland. At 10°C, the Antarctic octopus pump is around four times more active than the temperate version, previous experiments have shown.
To reveal why, Holmgren and his colleagues injected the RNA message that codes for the Antarctic and temperate octopus enzymes into the unfertilised eggs of a Xenopus frog. They tweaked the amino acid sequences to make chimeras more or less like the Antarctic enzymes.
The enzyme in the Antarctic cephalopod had previously been found to differ from the warm water one in 35 out of 1028 amino acids. Just 12 mutations allowed the temperate enzyme to replicate the cold tolerance of the Antarctic enzyme. Surprisingly, a single amino acid change allowed substantially higher activity in the face of extreme cold – switching a valine for a leucine at the interface of the protein and the lipid.
This single amino acid switch makes the opening of the ion pump more hydrophobic, so that it slips more easily into the cell’s membrane. ‘The amino acid made the protein greasier,’ says Holmgren. ‘The more greasy, the lower the energy required to move from one conformation to another and the faster the pump.’
Enzymes adapt to the cold by becoming ‘floppier’, so they need less energy to undergo conformational changes, something that is necessary when there is less thermal energy around, says Levental. ‘Membrane proteins have an added challenge of being in a lipid environment that is itself affected by cold,’ he adds.
The results ‘suggest that non-specific, physical interactions between proteins and lipids – rather than protein folding – are very important for cold adaption’, says Levental. ‘This is cool because it shows how important the subtle interactions between transmembrane domains and their solvating lipids can be.’
Enzyme cold adaptation is a much more interesting problem than heat adaptation, says Johan Åqvist, a biochemist at Uppsala University, Sweden and chair of the Nobel committee for chemistry. This latest research is impressive, he adds, and similar to his report on a globular enzyme, which found that the protein surface facing the solvent is mostly responsible for cold adaptation. He also notes that the engineered pump’s activation enthalpy has been reduced, just as in normal cold-adapted enzymes.
Though speculative, cold tolerance in enzymes may one day be important for cryogenic medicine or preservation or for organ transplantation, suggests Levental. ‘It appears to me that maybe the principles for adaptation of membrane-bound enzymes are not very different from regular enzymes.’
References
G Galarza-Muñoza, J Åqvistb and B Olav Brandsdal, Proc. Natl. Acad. Sci. USA, 2023, 120, e2301207120 (DOI: 10.1073/pnas.2301207120)
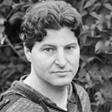
No comments yet