As global energy demands surge, the need for sustainable energy solutions is becoming more pressing. India’s ambitious goal of achieving 500GW of non-fossil energy capacity by 2030 (up from around 200GW today) is a cornerstone of the country’s strategy to combat climate change.1 As highlighted by India’s Panchamrit goals at COP26, a key component of this goal and reducing the country’s carbon intensity by 45% from 2005 levels, will be technologies that can store the energy produced by renewable energy sources and deliver it on demand.
While lithium-ion batteries (LIBs) have long dominated the energy storage market, their dependence on scarce resources like lithium and cobalt poses significant challenges in terms of cost and availability. India has almost no lithium deposits of its own and the high cost and geopolitical uncertainties surrounding lithium and cobalt extraction have led to supply chain problems for LIBs, which has slowed the transition to renewable energy. 2,3
To enable India’s self-reliance (Atmanirbhar) in energy storage, sodium-ion batteries (SIBs) are emerging as a promising energy storage alternative. The technology has comparable electrochemical properties to LIBs, but by using more abundant materials it offers greater scalability and lower manufacturing costs.4,5
However, developing cost-effective, high-energy-density sodium-ion batteries still poses a number of challenges, largely owing to the larger size and mass of sodium ions compared to lithium.6 While sodium-ion batteries are still in the early stages of commercialisation, they have already found applications in grid-scale energy storage systems. In 2023, a 2-hour 5MW/10MWh grid battery using sodium-ion technology was installed in China.
The challenges of sodium ion technology
While sodium itself is abundant, finding efficient,high-performance electrode materials remains a significant hurdle in realizing the full potential of SIBs. Achieving high energy density in SIBs is challenging due to their greater mass compared to LIBs, limiting their effectiveness in demanding applications like electric vehicles. Hard carbon is being used for full cell SIBs, but there is also need to explore more efficient anode materials to understand their diffusion kinetics.7,8 Cathode materials currently used in SIBs are mainly layered, polyanionic and Prussian blue analogues, which have many advantages but still need significant improvement in terms of energy density, cycle life and charging speed.9 Layered cathode materials offer better energy density, but these suffer from structural instability and rapid capacity fading, limiting their long-term viability.10,11,12 Therefore, polyanionic cathode materials such as NASICON compounds, including the promising Na₃V₂(PO₄)₃, are have been explored in recent years; however, these materials have low electronic conductivity, restricting their practical use in high-performance batteries.5
Potential solutions
The key to unlocking the potential of SIBs therefore lies in developing novel cathode materials. Specifically, cathodes with high energy density can be achieved through meticulous structural design and morphology control, ensuring optimal electrochemical performance and reversibility. Promising research is focused on synthesising nano-structured polyanionic materials, particularly manganese, and iron-based NASICON-type structures, due to availability of the materials, their enhanced oxidation stability, finely tuned operating potential and robust 3D framework.
Techniques such as nanostructuring, carbon coaring, doping and hybrid material systems have been widely adopted in lithium-ion battery research, leading to significant performance enhancements. Polyanionic manganese materials are particularly attractive because this element is abundant and cost-effective in India, offering significant advantages over materials such as vanadium and cobalt. However, manganese-based cathodes suffer structural instability due to Jahn–Teller distortion and low electronic conductivity, which hinder sodium-ion kinetics during cycling,9 and the lower redox potential of Fe3+/2+, which results in reduced energy density.10 Research is therefore focused on doping strategies to enhance structural stability during cycling and tuning the PO4 environment with more electronegative species, such as SO₄, to increase the operating potential through the inductive effect.13
Exploring alternatives to conventional polyolefin-based separator materials can also improve SIB’s efficiency and cost, for example using cellulose.14 With a new design of mixed polyanionic it is possible to achieve around 155Wh/kg energy density;13 however, the objective is to achieve an energy density exceeding 270Wh/kg, with a cycle life of at least 2000 cycles and 80% capacity retention.
Structural and political factors
As well as these technical and scientific barriers, government policies promoting renewable energy and clean technology are also needed to support the development and adoption of SIBs. Incentives for research and subsidies for green manufacturing processes are crucial enablers in this transition.
Advances in analytical techniques, such as in-situ measurements (XRD, XPS, EXAFS, Raman), are also needed for better understanding and optimisation of cathode materials. There is one synchrotron facility already available at RRCAT in India, however, we need to establish more such facilities to help in understanding the structural and interfacial evolution of the electrode materials.
The investment required for R&D and scaling up production processes can be a significant barrier, particularly for smaller companies and startups in the battery sector. The Indian government has recently announced roadmap to support the Indian energy storage industry (though few of the initiatives focus on SIBs specifically). Partnerships between industry players, research institutions and government bodies can not only facilitate knowledge sharing but also resource pooling and easy commercialisation of sodium ion batteries.
Successful collaborations between government, academia, and industry in the lithium-ion sector can serve as a model for developing sodium-ion battery technologies. For instance, partnerships in the development of solid- state batteries have accelerated innovation and commercialization timelines. The Faraday Institution in the UK, which brings together researchers from universities and industry, is an example of such a collaborative model.
As with any battery technology, the disposal of SIBs presents environmental challenges. The development of effective recycling methods is crucial to minimizing the environmental impact of battery waste. robust recycling infrastructures and creating economic incentives for recycling can help manage the disposal of SIBs. While SIBs may reduce the dependency on scarce and toxic materials, ensuring their end-of-life management is equally important for sustainable development.
By developing high-performance SIBs, the industry can overcome these barriers, accelerating the transition to cleaner energy sources and supporting India’s ambitious energy targets.
References
1 Y Singh et al. Heliyoni, 2022, 8, e10013, DOI: 10.1016/j.heliyon.2022.e10013
2 C P Grey and D S Hall, Nat. Commun., 2020, 11, 6279 (DOI: 10.1038/s41467-020-19991-4)
3 C Zhang et al., Adv. Funct. Mater., 2024, 34, 2308001 (DOI: 10.1002/adfm.202308001)
4 A Innocenti, S Beringer and S Passerini, Nat. Rev. Mater., 2024, 9, 347 (DOI: 10.1038/s41578-024-00657-2)
5 K M Abraham, ACS Energy Lett., 2020, 5, 3544 (DOI: 10.1021/acsenergylett.0c02181)
6 S K Sapra et al., WIREs Energy & Environment, 2021, 10, e400 (DOI: 10.1002/wene.400)
7 M Chandra et al., Electrochimica Acta, 2020, 331C, 135293 (DOI: 10.1016/j.electacta.2019.135293)
8 M K Singh et al., Chemical Engineering Journal, 2023, 454, 140140 (DOI: 10.1016/j.cej.2022.140140)
9 V K Tiwari and R K Singh, Chemical Engineering Journal, 2023, 471, 144592 (DOI: 10.1016/j.cej.2023.144592)
10 J Pati et al., J. Mater. Chem. A, 2022, 10, 15460 (DOI: 10.1039/D2TA01775J)
11 A Sengupta et al., Energy Storage Materials, 2024, 69, 103435 (DOI: 10.1016/j.ensm.2024.103435)
12 B S Kumar and A Mukhopadhyay, J. Power Sources, 2024, 610, 234716 (DOI: 10.1016/j.jpowsour.2024.234716)
13 J Pati and R S Dhaka, J. Power Sources., 2024, 609, 234646 (DOI: 10.1016/j.jpowsour.2024.234646)
14 S K Sapra et al., J. Mater. Chem. A, 2024, https://arxiv.org/abs/2409.06743
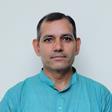
No comments yet