The revolutionary idea of using light as a reagent in reactions has arrived
The holy grail paper with perhaps the most intriguing title was written by a group led by the University of California, San Diego’s Kent Wilson. Wilson, who passed away five years after the paper was written, was known as a brilliant and unconventional scientist. His research spanned many different fields, but in his Accounts of Chemical Research article Wilson put forward ‘controlling the future of matter’ as chemistry’s greatest prize. The concepts linked to this lofty sounding goal generally belong to the field of ‘quantum control’ – essentially using light to control the behaviour of atoms and molecules. The hope was that specially shaped laser pulses could replace chemical reagents, directing the breaking and formation of chemical bonds.
I think this whole idea is remarkable and still has a vivid life
YiJing Yan, University of Science and Technology of China
‘This is really just a gorgeous idea and starts with thinking about how people can use an external field to regulate molecules and to guide them towards a desired reaction,’ explains YiJing Yan, a physical chemist at the University of Science and Technology of China, who worked as a postdoc in Wilson’s group in the early 1990s and was one of the holy grail’s authors.
Photonic reagents
Most chemists will be familiar with using molecular properties like leaving groups and sterics, or conditions like temperature and pressure to direct chemical reactions. Instead, Wilson’s group wanted to exploit molecules’ quantum nature to select which pathway a reaction would follow. Molecules are quantum objects – behaving both as particles and waves – and the interaction of light waves with molecules’ wave functions can be manipulated to steer chemical events.
Yan explains that the key theoretical grounding for the field was developed in the 1980s by researchers in the US and Israel. David Tannor and Stuart Rice at the University of Chicago and Ronnie Kosloff from the Hebrew University of Jerusalem explained how pairs of time-delayed laser pulses could be used to exert control over chemical reactions. Meanwhile, Moshe Shapiro and Paul Brumer then at the Weizmann Institute of Science, Rehovot, suggested exploiting the interference between photons of different wavelengths from two different lasers.
Another of the area’s most prominent researchers was Princeton University’s Herschel Rabitz. ‘He brought in a lot of engineering control theory combined with basic science, and introduced the idea that the photon is a type of chemical reagent,’ recalls Yan.
Rabitz himself points out that the field’s origins date even further back – ever since the invention of the laser, chemists have dreamed of using controlled light pulses to manipulate matter. A key milestone was the creation of ultrafast lasers that operate at timescales relevant to energy transfer within molecules. Rabitz likens earlier experiments to throwing a glass against a wall. ‘It breaks,’ he says. ‘But there was no way of controlling how it broke… And so people were firing picosecond laser pulses at molecules – they would break, but there was no way of altering the fragmentation pattern… They had energy and that was basically it.’
Trick of the light
In the mid-1980s, femtosecond lasers finally gave chemists the tools they needed, but these alone still weren’t adequate to fully control molecules’ behaviour. ‘The original lasers were one colour, but that’s equivalent to trying to play a Beethoven sonata with one key on the piano,’ says Rabitz.
Working alongside mathematician colleagues, Rabitz realised that ‘optimal control’ principles from engineering could help design the best light pulses for applications in chemistry. ‘From that early work we found that the optimal solution to get energy from here to there, or possibly break a bond, was a highly complex wave form of the electric field,’ he recalls. ‘But this had a negative response in the laser community because when I showed this to laser scientists, they said: “This is not a laser – lasers are red, or blue, or green.” They weren’t all these colours at once.’
By chance, the techniques required to shape light in this way were created around the same time by engineers in the telecommunications sector. ‘They wanted to use optical fibres to carry information between cities … and they recognised that shaping radiation carries information,’ says Rabitz. ‘So they developed the tools to shape ultrafast radiation pulses. And when we saw that we recognised this was, coincidentally, exactly what was necessary for meeting quantum control goals.’
The first pulse shapers used an optical grating with 128 liquid crystal pixels that could modulate the phase and amplitude of broad bandwidth laser light. Now, Rabitz’s optical ‘piano’ had 128 keys and, with the help of a computer, each key could be retuned hundreds of times a second.
The next problem was calculating what shape the laser pulses would need to take on for complicated molecular reactions. This would require solving the time-dependent Schrodinger’s equation and defining the system’s Hamiltonian – which, for anything other than the very simplest chemical systems, just wouldn’t be possible.
In the early 1990s, Rabitz proposed using software based on a learning algorithm to help steer the system. ‘What we suggested was: forget the Hamiltonian – we don’t know it,’ he says. The idea would be to apply a trial pulse to a sample and measure the response, for example by analysing the proportions of different products formed. Based on this, the algorithm would retune the laser pulse to guide the experiment towards a desired outcome.
‘What we said at the time was that the molecule is actually replacing the digital computer – it’s now an analogue computer and it knows its own Hamiltonian perfectly and solves its own Schrödinger equation impeccably,’ says Rabitz. ‘Just let it go around the loop and home in.’
Closing the loop
In the late 1990s, Wilson’s group became the first to put the idea into practice. Around the time Wilson wrote the holy grail paper, Rabitz went to meet him at his office in San Diego. ‘I asked him would he try this? Because he was capable of trying this as he had the resources. And he gave me a barrage of answers – he’s busy with this and busy with that… But the truth is in 1997 he actually did the very first experiment of this type,’ recalls Rabitz.
Using a computer-controlled pulse shaper, Wilson’s team optimised laser signals to efficiently induce fluorescence in a solution of dye molecules. It showed that the closed-loop principle worked.
Wilson’s experiment was soon followed by a team from the University of Würzburg in Germany. This group, led by Thomas Baumert, working in the laboratory of Gustav Gerber, performed controlled fragmentation experiments on iron complexes using an automated feedback system to steer the reaction towards chosen chemical products.
The field grew substantially after these early demonstrations, with numerous groups applying the techniques in hundreds of different experiments and thousands of models. Rabitz, who had previously focused on theory also got in on the act, setting up his own lab around the turn of the century. In one example, his team used a family of shaped light pulses to trigger similar dissociation reactions across a series of halomethane substrates. The study illustrated how photonic reagents can display regular reactivity trends akin to those seen in homologous series of traditional chemical reagents.
The shape of the problem
In the early 2000s, as successful experiments multiplied, one thing puzzled Rabitz – why did they all work? A standard pulse shaper could make 30128 different pulse shapes – a figure Rabitz notes is larger than the number of atoms in the universe. Despite this huge search space, optimal controls were repeatedly discovered within an hour of automated searching. Why did none of the attempts lead to a dead end?
‘It took us a year to go through this … and we came to the conclusion that there were no traps on the landscape to stop a search from fully reaching the quantum control objective. None. Zero. None whatsoever,’ says Rabitz. ‘I actually didn’t believe it at first – how could this be true?’
After contemplating this for a couple of weeks, it dawned on Rabitz that this idea that there were multiple solutions for every molecule explained everything. ‘This is why the simulations always worked and the experiments worked. There was an amazing piece of serendipity buried in quantum mechanics,’ he says. In the years since, Rabitz has continued to probe the underlying principles and believes that beyond quantum control, the finding could have important implications for other areas of science unrelated to quantum mechanics. ‘These are questions hard to turn away from,’ he says. ‘Time will tell us the answers and their full significance.’
Another major milestone was achieved in 2015, when a group from Germany and Israel, including Kosloff, used shaped laser pulses to control bond formation in a bimolecular reaction. In this example, magnesium dimers were generated by exposing a gaseous sample to femtosecond laser pulses. While bonds had previously been formed in laser-controlled intramolecular rearrangements, bringing photons together with two separate starting materials to make a new bond is an even more complex task. The University of Kassel’s Christiane Koch who worked on the project, told Chemistry World at the time that the group had initially found it hard to source funding for the project because reviewers simply ‘did not believe that the process could work’.
Branching out
Beyond the fascinating fundamental insights these experiments provide, Rabitz suspects that real applications in chemical synthesis, like those Wilson alluded to, are likely to be limited. ‘These are very expensive light sources,’ he says. ‘So you have to ask a basic question: what does a mole of these smart photons cost?’ The answer, Rabitz notes, doesn’t compare favourably to typical laboratory reagents. Unless the price of the exceptionally expensive titanium–sapphire lasers comes down substantially, he believes that quantum control methods are more likely to find uses in areas where cost is less of a concern.
Over the last two decades the field has branched out. A great deal of time (and money) has been invested in applying quantum control principles in the field of quantum computing, for instance. Another area where Rabitz feels these techniques can make an impact is in biological research. The techniques have been used to control the energy flow in a light-harvesting biomolecule involved in photosynthesis and, in another example, it was used to manipulate photoisomerisation reactions in a retinal protein. Rabitz too has shown how quantum control can discriminate between almost identical flavin molecules that are indistinguishable using conventional spectroscopic tools. This suggests a use for shaped laser systems in molecule-selective bioimaging.
Twenty-five years after contributing to the holy grail paper, and despite his own research moving in a slightly different direction, Yan reflects that quantum control is still just as exciting. ‘I think this whole idea is remarkable and still has a vivid life,’ he says. As researchers from other fields begin to make use of quantum control principles, he believes these techniques will contribute towards even bigger goals. ‘I would still say now, it’s a whole philosophy that gets richer and broader each year.’
Top 20 caption: Here we can see the 20 most-cited papers related to quantum control that have been published since 1995. The Assion and co-workers paper in third place, with 1382 citations, is the 1998 report on Thomas Baumert and Gustav Gerber’s first experimental use of closed-loop optimal control in bond breaking. The R J Levis paper in sixth place also used closed-loop optimal control, in this case to optimise intramolecular rearrangement reactions in a series of organic molecules. The J L Herek paper with 549 citations was the demonstration of quantum control to manipulate energy flow in a light-harvesting biomolecule (LH2).
Co-authors caption: Here we see researchers who have published impactful papers on quantum control, linked to their co-authors. The size of each point reflects the number of highly-cited papers published by each author, while the colour denotes when their first key publication in the field was published, going back as far as 1995. Many of the key groups referenced in this story are easy to find in discrete clusters. Herschel Rabitz is the largest node near the bottom of the image, while Kent Wilson appears just to the left of centre. Paul Brumer and Moshe Shapiro who contributed important early papers on coherent control can be found about half-way between Wilson and the leftmost edge of the image. Gerber and Baumert are nearer the top of this chart.
Cite-network caption: This chart links researchers to other researchers who cite their work. Most of the key characters in the quantum control story are found in the large cluster to the left of the image, including Rabitz, Shapiro, Wilson, Gerber and Rice. Moving further right in the network we find many more researchers with interests in quantum computing and quantum information sciences.
Geo caption: This map shows the key centres of quantum control research in the US, Europe and Israel. The line along the bottom shows the number of highly cited papers published each year, clearly showing the growth in interest in the field through the 2000s.
Additional information
For an explanation of how these data visualisations were built, read Behind the data
Further reading
1 X Wang et al, Harnessing deep neural networks to solve inverse problems in quantum dynamics: machine-learned predictions of time-dependent optimal control fields, Phys. Chem. Chem. Phys., 2020, DOI: 10.1039/d0cp03694c
2 A Szokeet al, Entangled light–matter interactions and spectroscopy, J. Mater. Chem. C, 2020, 8, 10732 (DOI: 10.1039/d0tc02300k)
3 J Chen et al, Ultrafast photofragmentation of Ln(hfac)3 with a proposed mechanism for forming high mass fluorinated products, Sci. Rep., 2020, 10, 7066 (DOI: 10.1038/s41598-020-64015-2)
4 U Kumar et al, Single plasmon spatial and spectral sorting on a crystalline two-dimensional plasmonic platform, Nanoscale, 2020, 12, 13414 (DOI: 10.1039/d0nr02066d)
5 M Y Livshits et al, An excited state dynamics driven reaction: wavelength-dependent photoisomerization quantum yields in [Ru(bpy)2 (dmso)2]2+, Chem. Sci., 2020, 11, 5797 (DOI: 10.1039/d0sc00551Gg)
6 G Tao, Topology of quantum coherence networks in singlet fission: mapping exciton states into real space and the dislocation induced three dimensional manifolds, Phys. Chem. Chem. Phys., 2020, 22, 1258 (DOI: 10.1039/c9cp05102c)
7 M Sindhal et al, Quantum-nondemolition state detection and spectroscopy of single trapped molecules, Science, 2020, 367, 1213 (DOI: 10.1126/science.aaz9837)
8 S Falcinelli, Quantum-state controlled reaction channels in chemi-ionization processes: radiative (optical–physical) and exchange (oxidative–chemical) mechanisms, Acc. Chem. Res., 2020, DOI: 10.1021/acs.accounts.0c00371
9 H Agueny, Quantum control and characterization of ultrafast ionization with orthogonal two-color laser pulses, Sci. Rep., 2020,10, 239 (DOI: 10.1038/s41598-019-57125-z)
10 N W Hendrickx et al, A single-hole spin qubit, Nat. Commun., 2020, 11, 3478 (DOI: 10.1038/s41467-020-17211-7)
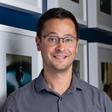
Searching for the holy grails of chemistry
- 1
- 2
- 3
- 4
- 5
- 6
Currently reading
How lasers could replace chemical reagents – for a price
- 7
- 8
- 9
1 Reader's comment